WHITE PAPER
Ultra-Stable Femtosecond Amplifier Enables High Data Output in 2D IR Spectroscopic Studies of Biological Membranes and Surfactants
Overview
Researchers in the laboratory of Prof. Carlos Baiz (University of Texas, Austin) are investigating the dynamics of hydrophilic/ hydrophobic interfaces to understand the detailed function of both biological membranes and industrial surfactants. They make extensive use of a two-dimensional infrared spectroscopy set-up based on an Astrella ultrafast laser amplifier, where the combination of output stability and operational simplicity has enabled high lab throughput as measured by publishable data
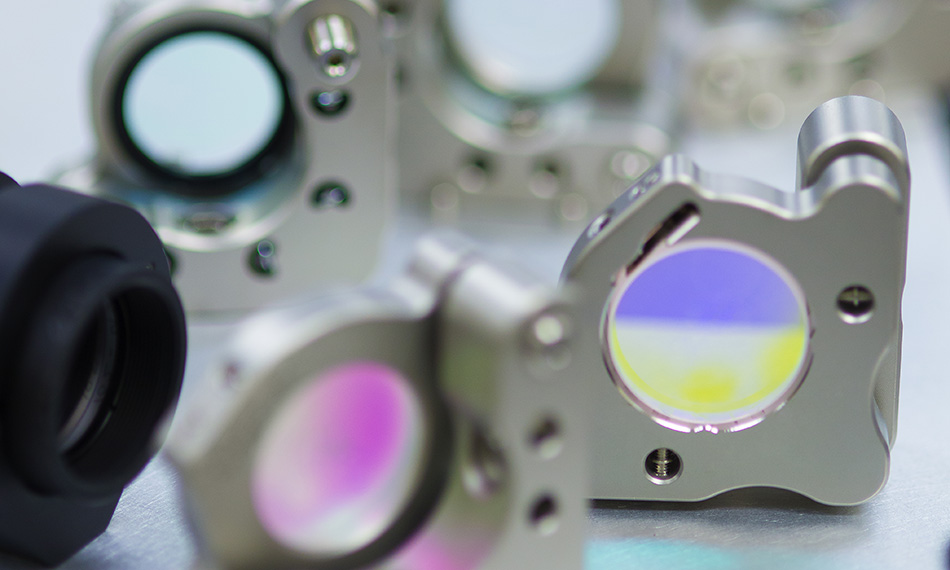
Real-world membranes and industrial surfactants
Professor Baiz explains the motivation of his research, “Traditional research in both surfactants and lipid membranes has targeted simple model systems where a single type of lipid or surfactant species is involved. While this has provided important information, this understanding is not always reflective of real-world systems. For example, biological membranes contain hundreds or even thousands of different lipids. Now we know that biology naturally tends to efficiency, so this unexpected chemical diversity undoubtedly serves an important purpose. In our research, we examine the role of membrane heterogeneity on the folding of certain proteins that control cellular function.” He adds that similar chemical diversity is also found in the world of industrial surfactants that are used for applications like oil recovery and lubrication. Whether these are derived from petrochemical sources or biological sources like palm oil, the molecules are characterized by many differences in terms of chain lengths, functional groups, branching and so forth.
"The principles of 2D spectroscopy are well understood, but the implementation has often been quite complex."
The unique power of 2D IR spectroscopy
2D IR spectroscopy is one of several powerful tools the Baiz group use in their research. The principles of 2D spectroscopy are well understood, but the implementation has often been quite complex. In a conventional (one-dimensional) infrared absorption measurement, the amount of absorption is measured as a function of a single parameter, the infrared frequency, usually employing a FTIR (Fourier Transform) spectrometer. Each absorption peak corresponds to a different molecular vibration. In 2D IR, the absorption is effectively measured as a result of two infrared sources – called the pump and probe. The data is then typically plotted as a 2D contour map in the two frequencies, where the diagonal plot is just the linear spectrum. As shown in Figure 1, false color is used to represent signal intensity in these data plots. [1]
Why? What does this rather complex experimental approach deliver that you can’t get easily by other means? The presence of off-diagonal peaks tells you that those two vibrations are coupled – either sharing one or more atoms, or closely connected in some type of chemical interaction. For example, the shape of the peaks reveals the homogeneous and inhomogeneous broadening components and this—in turn—provides dynamic information on the interaction of molecules with their nearby environment. Other more subtle information can also be extracted from the data set through analysis and modeling.
While the data is plotted in the frequency domain, today most 2D spectroscopy is performed in the time domain using ultrafast laser pulses, as shown schematically in Figure 1. The sample is excited by two broadband femtosecond pump pulses containing all the frequencies of interest. The delay between the two pump pulses is then repeatedly scanned and the resulting impact on the probe pulse is Fourier-transformed to the frequency domain. (It’s a conceptually similar to the way FT-NMR is performed). The probe pulse is also a broadband femtosecond pulse containing all the frequencies of interest. After this passes through the sample it is then dispersed on a monochromator to directly obtain the probe frequency axis. Since spectra are recorded using laser pulses, the delay between the pump and probe can also be controlled to show the temporal behavior, e.g., coherence time, of all these effects.
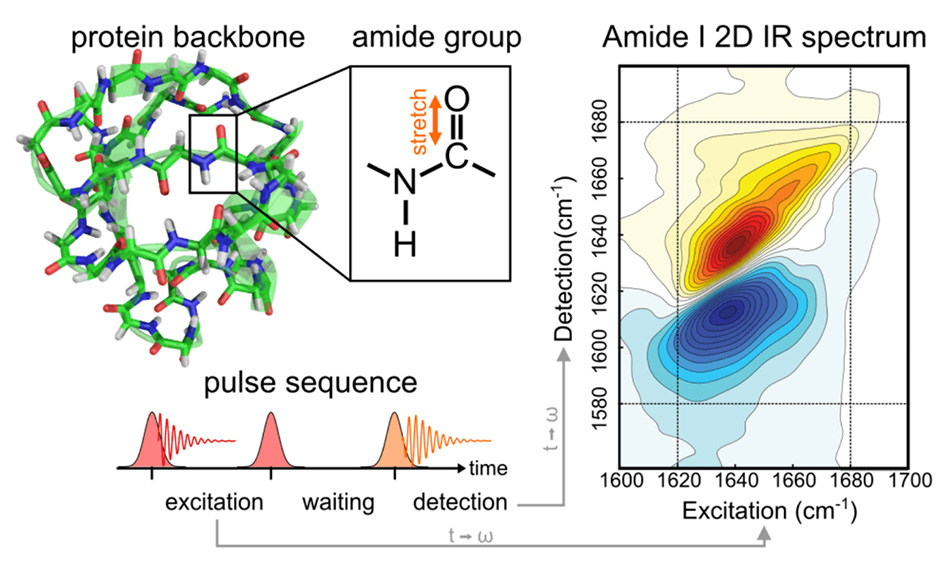
Figure 1: In 2D IR spectroscopy, a pair of broadband mid-infrared pump pulses cause a vibrational coherence in the sample. After a brief delay, a third broadband pulse is applied to generate the measured third-order signal. Figure courtesy the Baiz lab.
A robust experimental system with multiple capabilities
Baiz notes, “2D IR is well-proven to be an excellent method to probe the dynamics of hydrogen bonding in lipid membranes. The hydrophilic/hydrophobic difference is what defines the interface, and hydrogen bond dynamics are highly disrupted at the interface compared to the bulk.” Baiz adds that another advantage of 2D IR for his studies is the relatively strong signal that can be obtained from carbonyl (C=O) stretching vibrations. The majority of common lipids have these carbonyl groups which are key participants in the hydrogen bonding with water, so it really is a great match for his work targeting lipids in various heterogeneous ensembles. [2-3]
The laser source in the 2D IR setup is a Coherent Astrella in conjunction with a tunable optical parametric amplifier (Coherent TOPAS Prime with NDFG). The Astrella is a unique one-box amplifier generating 100 fs pulses at a center wavelength around 800 nm. The OPA/NDFG setup produces pulses tunable across the entire mid-infrared. It is usually tuned to a center wavelength between 5.7 and 6.2 microns in the Baiz setup, which corresponds to a frequency of 1750-1580 cm-1 in order to match the carbonyl stretching vibrations in lipids and proteins. The 100 fs pulse width provides sufficient spectral coverage to probe lipid ester carbonyls and protein amide carbonyls during the same measurements.
"2D IR is well-proven to be an excellent method to probe the dynamics of hydrogen bonding in lipid membranes."
Thermometry with Simultaneous Space and Time Resolution
Figure 2 schematically shows the main elements of the 2D IR setup used in the Baiz lab. In the early days of ultrafast 2D IR experiments, the separate pulses were generated using custom-built equipment. They were created on different beam paths leading to a significant alignment challenge to overlap all the beams at the sample with interferometric quality. Today researchers like Baiz use a commercial pulse shaper (the Quickshape from PhaseTech Spectroscopy Inc.) to generate the closely spaced excitation pulses and all the pulses are produced on a single collinear beam path, greatly simplifying the experiments.
The advanced electronic pulse delay capabilities of the Coherent Astrella signal-delay-generator (SDG Elite) is useful for some of the more advanced experiments, such as transient-2D IR spectroscopy. In this mode, an additional UV laser is used to phototrigger chemical reactions which are then tracked via 2D IR. [4] Analogous to traditional “pump-probe” methods, this implementation can be thought of as “UV-pump-2D IR probe”. The multiple outputs of the SDG Elite are used to synchronize the different components of the optical setup as shown in Figure 2.
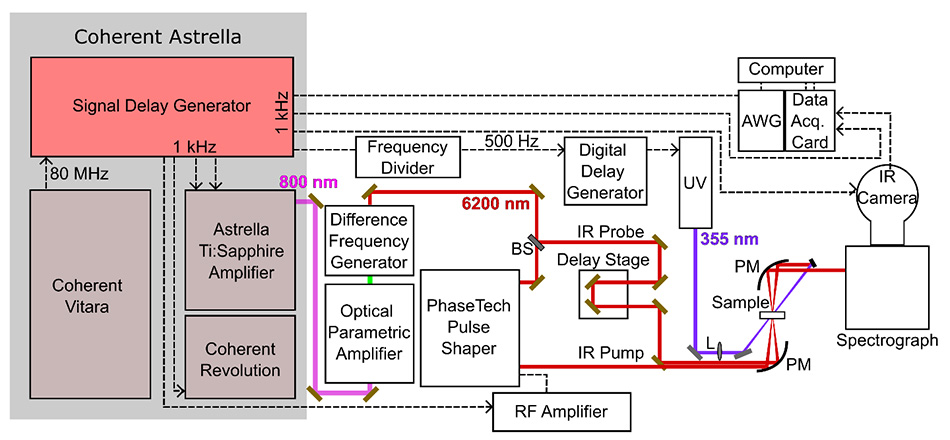
Figure 2: Schematic illustration showing the main elements of the 2D IR setup. The Coherent Astrella system includes the Vitara oscillator, Revolution pump laser, and the main Ti:Sapphire amplifier. The Signal-delay-generator is used to generate the electronic timing pulses to synchronize the setup. Figure courtesy the Baiz lab.
Laser stability enables long data acquisition times
The Astrella is designed and manufactured at Coherent using HALT/HASS protocols that have resulted in unmatched laser stability and reliability. HALT stands for Highly Accelerated Life Testing and HASS stands for Highly Accelerated Stress Screening. Baiz cites Astrella’s outstanding stability as a critical enabling factor in his studies.
That’s because many of the 2D IR experiments performed in the Baiz group often require data acquisition times of 16-24 hours per sample and every aspect of the laser output must remain completely stable during this entire time. The reason for the long data times is the small signal strength. He explains, “2D IR signals are weak since the oscillator strengths of IR transitions are typically much lower than electronic transitions. In addition, we use isotopic substitution; in order to target one lipid species in a heterogeneous mixture, we label that lipid with carbon-13 to shift its C=O stretch to a lower frequency. So if our target lipid represents say 5% of the total, that’s a further drop in signal strength. Then if we want to do time dependent studies, well the experiment gets even longer.”
Operational simplicity minimized pandemic impact in Baiz lab
The 2D IR setup is used as a shared resource by multiple students and post-docs in the Baiz lab, which makes the long term reliability and simple operation of Astrella just as important as output stability. Baiz explains, “Students get allocated exclusive use in time blocks of typically two weeks duration. For this scheduling to work smoothly and fairly, we need dependable on-demand performance. We get that with Astrella. In fact, we’ve found the best solution is to just leave the laser running continuously 24/7 and essentially forget about it. I also want to add that we also have a great service contract which gets us back up almost immediately on the rare occasions we do have any laser issue.” Baiz says that ease of operation of Astrella – “It’s really just a light source” – has always enabled his students to focus all their energies on their experients, and never on the laser. But this was particularly important for lab productivity during the 2020 pandemic restrictions due to Covid. He notes, “University restrictions meant that only one person at a time could work in our lab. So junior students with minimal existing experience using an ultrafast laser amplifier had to work with no direct help or supervision. Yet this staffing limitation had no drop-off in our lab productivity at all, with 10 papers submitted in 2020 alone.”
Unique information derived from these 2D IR studies
An example of some of the exciting new science revealed by 2D IR spectroscopy is illustrated in Figure 3 which shows the simulated details of a protein embedded in a lipid membrane. The traditional view was that water is generally excluded from the hydrophobic interior. But data from isotope-labeled 2D IR experiments have shown significant water penetration within the membrane core. This snapshot is from a molecular dynamics (MD) simulation of an amphiphilic peptide in a membrane based on this data. Note that even at depths of ~1 nm into the hydrophobic (acyl chain) region, water molecules can H-bond with the backbone. For visual simplicity, the surrounding lipids are shown here as semitransparent sticks and only water molecules surrounding the peptide are shown. The average lipid headgroup positions are indicated as dashed lines.
"University restrictions meant that only one person at a time could work in our lab. So junior students with minimal existing experience using an ultrafast laser amplifier had to work with no direct help or supervision."
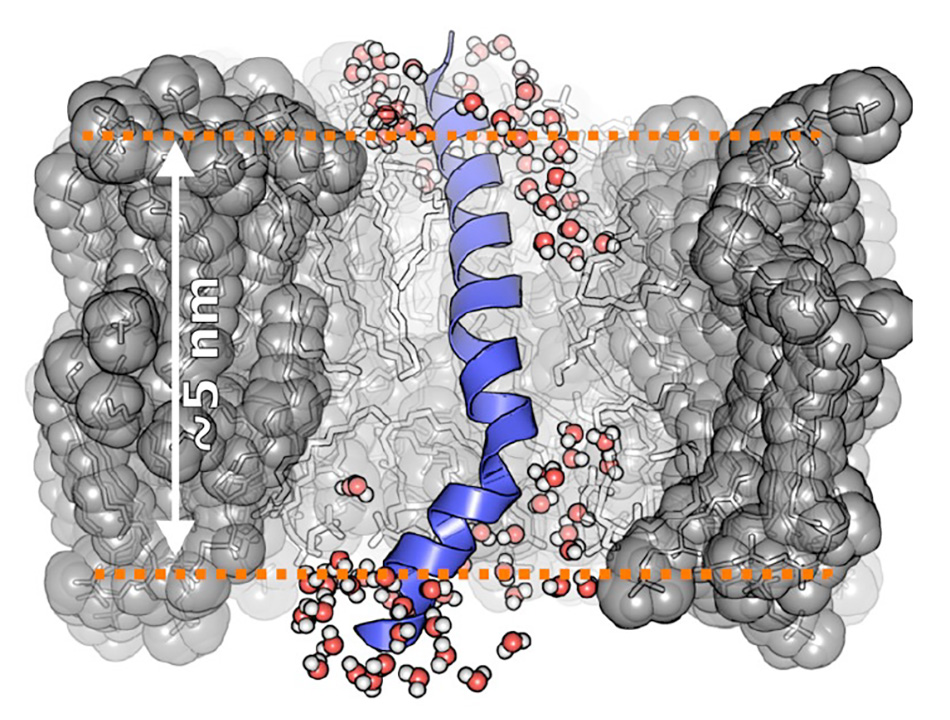
Figure 3: Water penetration into hydrophobic membrane environments.
Figure courtesy Baiz lab.
Summary
2D IR spectroscopy is a powerful technique for interrogating chemistry at the single-bond level. Advances in technical simplicity and system reliability, particularly in turnkey ultrafast laser amplifiers, now mean that research labs can use this as an on-demand analysis method, with the same accessibility and dependability as well-established routine methods like FTIR.
References
[1] Flanagan, Jennifer C., Mason L. Valentine, and Carlos R. Baiz. “Ultrafast Dynamics at Lipid–Water Interfaces.” Accounts of chemical research 53.9 (2020): 1860-1868.
[2] Flanagan, Jennifer C., Alfredo E. Cardenas, and Carlos R. Baiz. “Ultrafast Spectroscopy of Lipid–Water Interfaces: Transmembrane Crowding Drives H-Bond Dynamics.” The journal of physical chemistry letters 11.10 (2020): 4093-4098.
[3] Flanagan, Jennifer C., and Carlos R. Baiz. “Site-specific peptide probes detect buried water in a lipid membrane.” Biophysical journal 116.9 (2019): 1692-1700.
[4] Flanagan, Jennifer C., and Carlos R. Baiz.