WHITE PAPER
High stability laser source for cold atoms applications
Cold atoms research, which historically started as part of the atomic physics field, has grown into a wide, highly interdisciplinary research effort. Building upon developments of laser cooling and trapping [1] and the demonstration of a Bose-Einstein Condensate (BEC) [2], the field now involves atomic, molecular, and optical (AMO) physics, theoretical modelling, condensed matter physics, quantum chemistry, laser technology, and other disciplines. Thanks to the various magneto-optical techniques, the BEC can now be manipulated, probed, and investigated, providing a powerful tool for researchers working with multiple aspects of fundamental physics, atomic clocks, quantum information, sensing, metrology, and superconductivity.
Underpinned by a wide-ranging research, there is a strong focus worldwide [3 – 5] to create next-generation technological devices based on functional elements that use cold atoms. For example, the so-called “atomtronics” field is looking to create elements equivalent to the conventional electronic components, including diodes, transistors, and memory elements. The main building blocks in such efforts are typically BECs, trapping potentials and ways to move, manipulate, and detect atoms. Efforts are ongoing to create practical ultra-precise measuring and sensing devices (gravimeters, accelerometers, magnetic field sensors, etc.) as well as quantum information and computing elements by using building blocks enabled by the cold atoms community.
In this whitepaper, we discuss one of the important methods in the field of cold atoms – atom trapping, cooling, and manipulation by using far-detuned, off-resonant laser light. We review the techniques and main applications of the optical dipole traps, evaporative cooling, and optical lattices and discuss the stringent requirements these techniques put on the laser systems used. We also provide an insight into the technology behind Coherent’s ultra-low noise continuous-wave laser product line that is successfully used in the discussed applications.
Optical dipole trapping is a well-established technique allowing confinement of the cloud of cold atoms by using laser beams [6]. The atoms used are already cooled to mK – μK temperatures by using conventional Doppler cooling techniques before loading them into the trap. Such an optical trap is created when the oscillating electric field of a light wave induces an electric dipole moment in the atoms, which are therefore attracted or repelled by the extrema of the light’s intensity. The sign of the force depends on whether the frequency of light is lower (ωlaser < ωres, red-detuned traps) or higher (ωlaser> ωres, blue-detuned traps) than the atomic resonance frequency of the particular atom species.
The most simple dipole trap can be created by simply focusing a single red-detuned laser beam to increase the light intensity in a certain area of the experiment where atoms need to be trapped. Differently shaped traps can also be created by crossing multiple laser beams (Fig. 1). Blue-detuned light enable different forms of trapping potentials, such as “box” type traps.
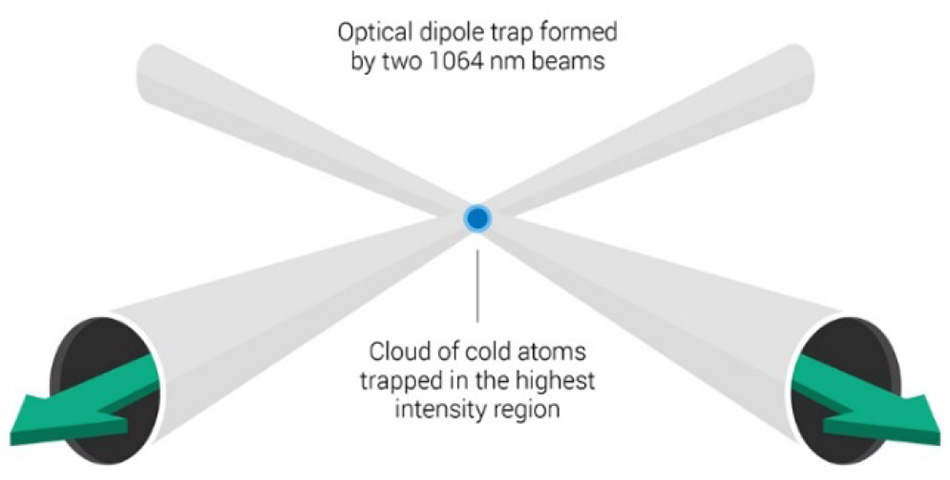
Fig. 1. Optical dipole trap
The optical dipole trap is a versatile method in cold atoms experiment. First, the loaded trap enables confinement of atoms in a defined space. It is then also possible to shift them from one part of the experiment to another. Being a conservative-type trap, it does not induce optical excitation and the forces only depend on the positions of the atoms.
Optical dipole traps played important role in the 80s and 90s when Doppler cooling techniques reached a lower limit at temperatures in the mK range. Going to lower temperatures was mainly prohibited by heating effects induced by photon scattering. The achieved temperatures were too high for creating atomic BEC. The evaporative cooling method was thus developed to further reduce the temperature of the atoms. The principle of this method relies on the potential trap which is created by off-resonant laser beams as discussed previously or by using inhomogeneous magnetic fields [6]. Once the atoms are confined, the height of the trap is reduced by controlling the laser intensity. The fastest (“hottest”) atoms then escape (“evaporate”) from the trap carrying kinetic energy away with them as the rest of the atoms re-thermalize at a lower temperature (Fig. 2). The height of the trap is reduced and the process is repeated until the atoms form a BEC. This method is now commonly used in one form or another in experiments where cold atom temperatures of few μK to nK are required.
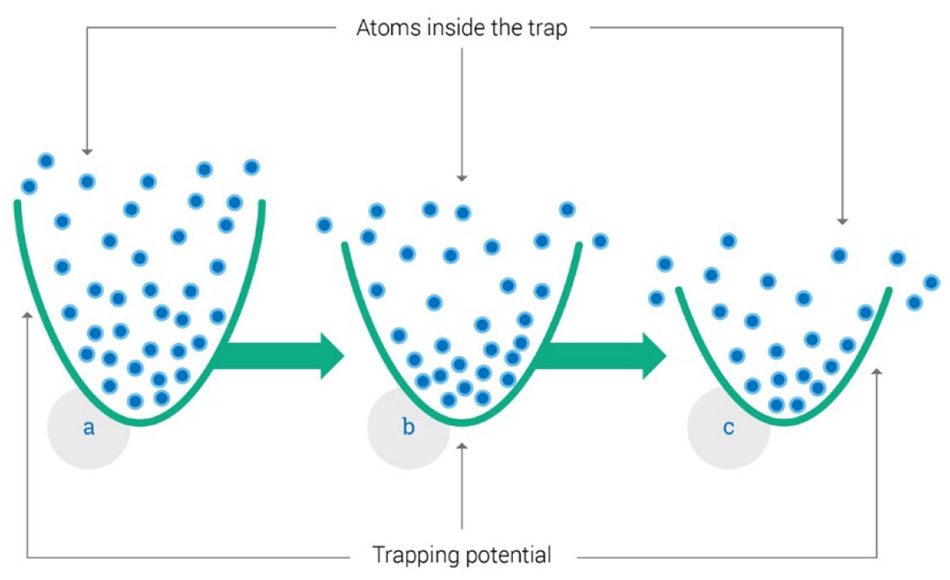
Fig. 2. Evaporative cooling
Another powerful tool that is provided by using off-resonant laser light are optical lattices. By using stable, off-resonant light, optical lattices are created by interfering multiple laser beams. Instead of “bulk” trapping in an optical dipole trap, an optical lattice provides many microscopic potential wells which are arranged in a periodic pattern (Fig. 3). Different shapes of such potential landscapes can be created by using red-detuned light, blue-detuned light, or a combination of both. 3D optical lattices can mimic the structure of a solid-state crystal at a much larger scale where light interference patters represents the crystal lattice and cold atoms mimic the electrons. Such defect-free, tunable lattices allow measurement times in the seconds range and serve as an investigative model to answer some key questions in solid-state physics. A few research highlights are mentioned in the non-exhaustive list below:
Phase transitions – working with ultracold atoms and being able to manipulate their states with optical lattice configuration and magnetic fields allows access to various quantum phases. Study of these states of matter, their properties, and transition dynamics are of great interests for researchers working with condensed-matter physics and superconductors. For example, reversible transitions from a superfluid BEC to a Mott insulator were thoroughly studied [7].
Atomic clocks – A key technology in metrology, atomic clocks were formerly based on microwave techniques. Optical atomic clocks have seen rapid development in the past decade, with optical lattice-based atomic clocks currently leading in stability and systematic uncertainty performance.
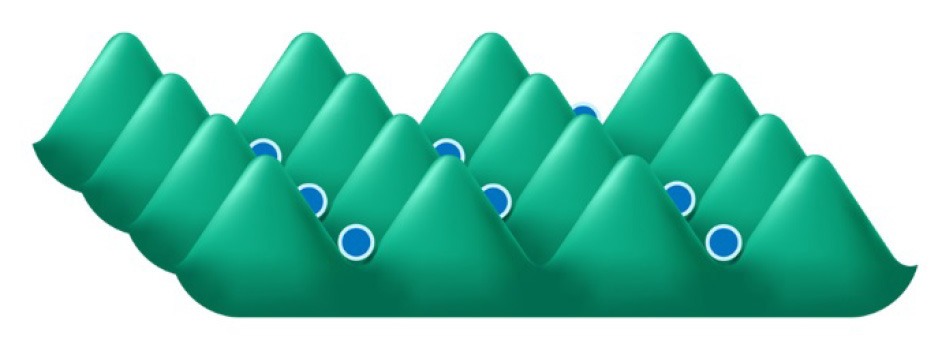
Fig. 3. Cold atoms confined in an optical lattice
Diatomic molecules – The majority of research to date has been done by cooling and working with a single atom type (typically neutral alkali type atoms featuring transition lines easily accessible with tunable laser sources in the cooling stage – Rb, Cs, Li, Na, K, but also more complicated structure atoms like Ca, Sr, Yb, Dy). Now there is also a growing interest in ultracold polar diatomic molecules which offer additional functionality. Such pairings might be created through a photo-association process or through Feshbach resonances. For the researchers studying quantum interactions, they offer a highly controllable way to study many-body phenomena and long-range dipole-dipole interactions when placed in optical lattices. Such labcreated ultracold diatomic molecules were demonstrated with Sodium-Potassium (NaK), Potassium-Rubidium (KRb), Lithium-Rubidium (LiRb) pairs. Homonuclear cold molecules were also created (K2, Rb2, Na2) using such techniques. In addition to this “synthetic” creation of cold molecules, large efforts are also put in direct cooling of molecules.
Quantum simulators – cold atoms in optical lattices provides means to design an experimental systems that could act as a model of certain question in quantum physics that are not yet accessible theoretically or numerically. Such simulators should have means to control the parameters of the experiment, to manipulate the atomic states, and to read out the outcomes. Previously discussed tools (like different quantum phases and diatomic molecules) would often be used in such experiments. Such simulator models could potentially provide insights into problems that cannot be solved by classical computing.
Laser Source Requirements
As the experiments discussed above are dealing with atoms in temperatures of micro to nano Kelvin, they are extremely sensitive to any noise sources which ultimately limit the resolution of the experiment or the measurement time. The laser system will inevitably influence this. Different laser systems might exhibit intensity noises originating from its pump diodes, relaxation oscillation noises, electronics control, and non-linear effects. Frequency noise (jitter in laser emission frequency) might be influenced by cavity thermo-mechanical properties. Also, the experiments might be more sensitive to some specific frequencies, like the trap frequency. In addition to the laser stability question, there are other factors to consider when buying a laser source for cold atoms work. Being a part of large and complex experimental setup, it is important that the laser will provide reliable day-to-day operation without needing to be readjusted or actively maintained. Any unplanned downtime in the experiment negatively impacts the throughput of the scientific research. Thus a reliable turn-key, easy-to-use system is desired.
For optical dipole and lattice cold atoms experiments, a continuous wave (CW), far off-resonant wavelength around 1μm is often chosen (for red-detuned traps). This wavelength provides enough spectral offset for most atoms to avoid any optical excitation and is conveniently available on the market from Ytterbium-doped solid-state and fiber lasers with output powers scalable up to tens of watts. High power is advantageous as it increases the depth of the optical dipole trap. Other important laser parameters include:
Laser linewidth – a narrow line single-frequency emission is essential for well-defined interference patterns. The measurement time of the laser linewidth must be taken into account when this parameter is specified.
Relative intensity noise (RIN) – the lowest possible noise is desired as any fluctuations in intensity will increase the heating rates of the cold atoms.
Frequency noise – the frequency noise of the laser will influence the heating rates of the atoms as well, thus the minimal fluctuations are desirable, especially if the laser in experiment is not frequency stabilized to external references.
Mephisto – lasers for the most demanding applications
Coherent’s approach towards CW high-stability laser sources is based on a non-planar ring oscillator (NPRO) technology. Since its invention in Stanford University [8], this technology has been recognized as the lowest-noise CW laser architecture available. It provides the basis for all Mephisto lasers, with the master oscillator cavity based exclusively on a monolithic crystal rather than discrete optical elements (Fig. 4). Such lasers deliver extremely low frequency and amplitude noise. Intrinsic linewidths of <1kHz over 100 of milliseconds are available due to laser’s extremely low phase noise. Furthermore, this narrow emission line can be tuned around its central emission frequency with high precision by adjusting the NPRO crystal temperature or by fine-tuning it with a fast integrated piezo transducer (PZT). It allows the user to have full control over the laser emission – which is extremely important in atom cooling and trapping experiments. This control also enables locking the laser to an external reference when even higher frequency stability is needed. For example, the user may wish to lock the laser to an external high-stability cavity or iodine line by having access to frequency control of the laser.
Apart from a narrow linewidth and low phase noise performance, important for creation of efficient interference structures, Mephisto products also provide low amplitude noise, which is further improved by Noise Eater (NE) technology. As in many diode-pumped solid-state or fiber lasers, pump diodes and the relaxation oscillations are the prominent intensity noise contributors. The Noise Eater effectively eliminates these two components by providing a feedback signal to the pump diodes. For a more detailed description of the technology behind Mephisto products, please see [9].
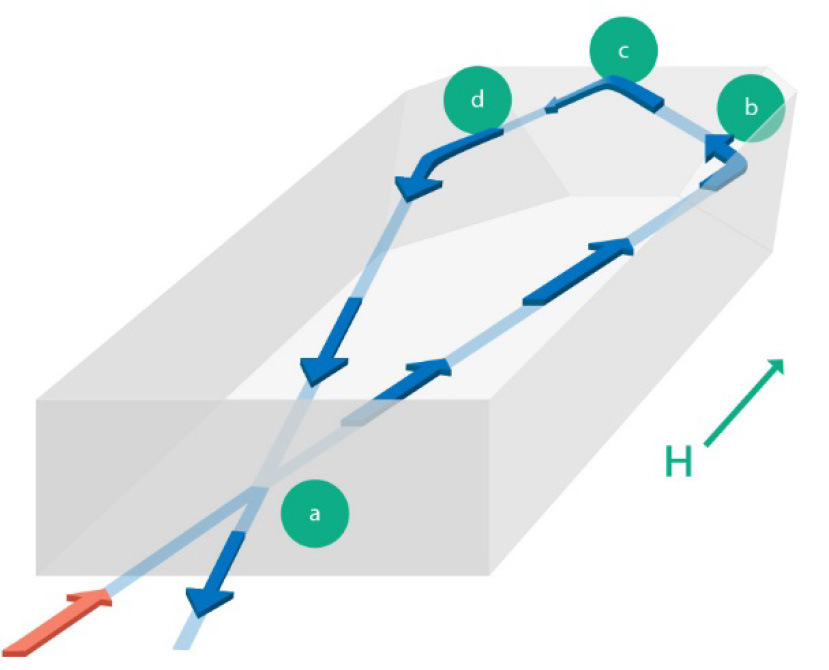
Fig. 4. Schematic representation of NPRO crystal. Orange arrow indicates the pump light and blue arrows show the path of the laser mode.
Due to its superior stability parameters, Mephisto is the laser of choice in some of the most demanding low-noise laser applications. This includes Gravitational Waves detection [10], interferometric measurements, low-signal heterodyning, metrology, and similar applications. Atomic physics experiments benefit especially from high-stability and high-power when off-resonant wavelengths are used to form sufficient depth of potential traps and high-precision stable optical lattices.
Output power straight from the NPRO master oscillator is commercially available up to 2W. At higher powers the laser performance might be compromised by transverse and longitudinal mode instabilities due to thermal effects. However, the applications discussed in this whitepaper require much higher powers, namely, tens of Watts, while retaining ultra-narrow linewidth, low noise, and high-frequency stability.
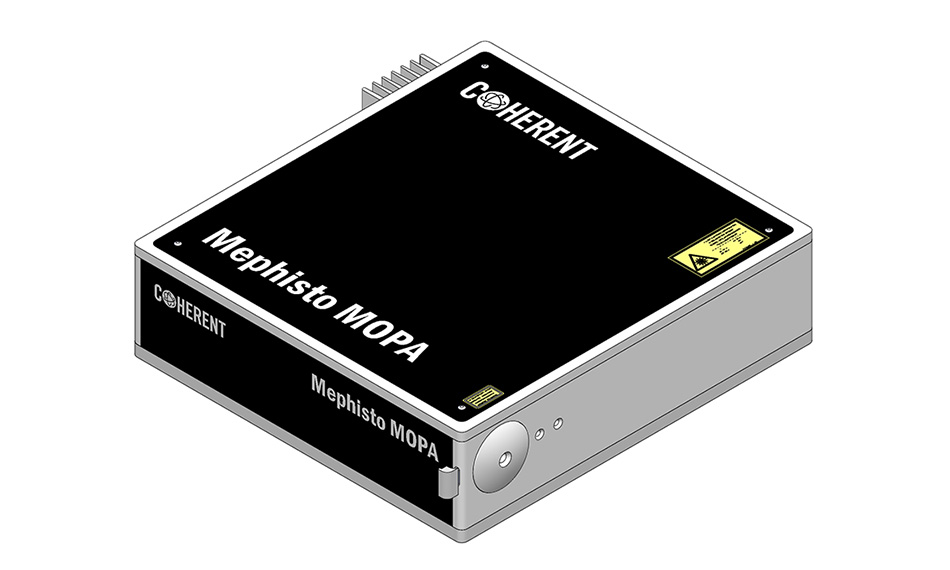
Fig. 5. Coherent Mephisto MOPA
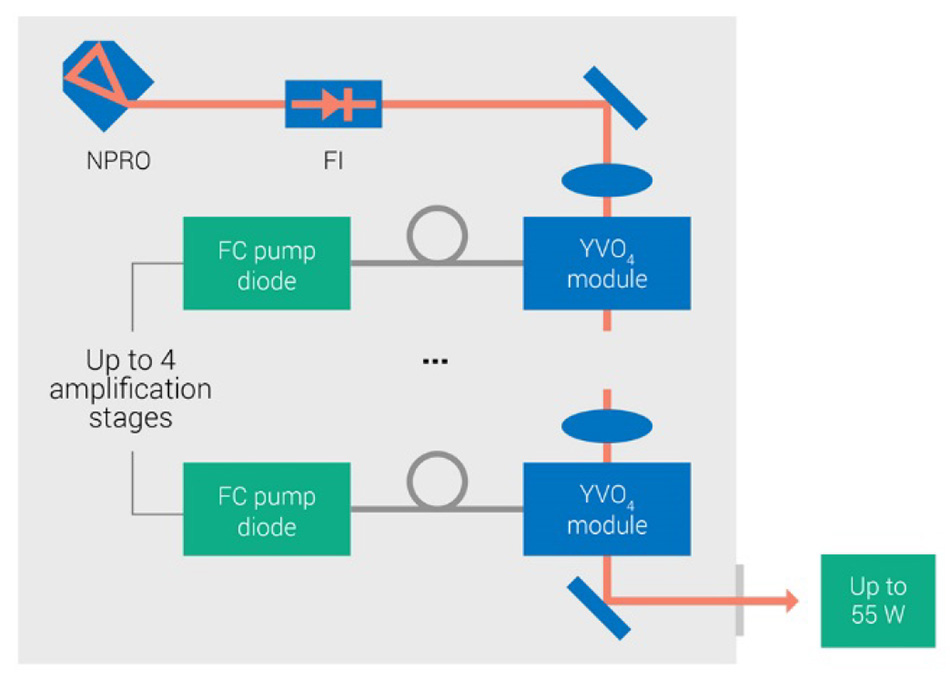
Fig. 6. Schematic representation of Mephisto MOPA design
To overcome this power limitation, Coherent uses a Master Oscillator Power Amplifier (MOPA) approach. The NPRO oscillator is used as a seed source in this configuration. The output of this seed laser is amplified gradually by using up to four amplification stages (diode pumped neodymium vanadate crystals, Fig. 6). Because of the MOPA configuration, the parameters of the laser are defined inside the seed laser, where the NPRO crystal is operated at an optimum power level.
The MOPA provides power amplification up to 55W at 1064nm with uncompromised stability of the standard Mephisto in a factory-integrated single-box solution. As a result, similar ultra-narrow linewidth, phase noise spectrum, and frequency tuning capabilities are available. Importantly to the cold atoms applications, the influence on the laser amplitude noise is minimal – no additional noise is added at the frequencies above 50kHz. A small increase at lower frequencies is created by MOPA control electronics (See Fig. 7). Using RIN spectrum, the laser noise-induced cold atoms heating rate in optical trap can be calculated [11] – Fig. 8. Due to low-intensity noise from Mephisto MOPA, the heating rate can be significantly lower as compared to other laser technologies. Good beam parameters and extremely long coherence length (>1km) makes the beam easy to manipulate in the experimental setup, especially where multiple beams are formed by beam splitters and retro-reflectors. Most of the scientific experiments using cold atoms are relatively complex, including tunable lasers, Zeeman slower, vacuum chambers, atom sources, related optoelectronics, etc. Thus it is important for us to provide a single-box solution which includes a fully integrated laser system and easy, turn-key operation – in this way, the user can focus on the experiment rather than the maintenance of the laser. In addition to Mephisto MOPA, which is based on all-solid-state technology, Coherent also offers CW single-frequency fiber amplifiers through the NuAmp product line. NuAmp products provide power amplification up to 50W, enable beam delivery by fiber, and are available in the wavelength range of 1030 – 1110nm.
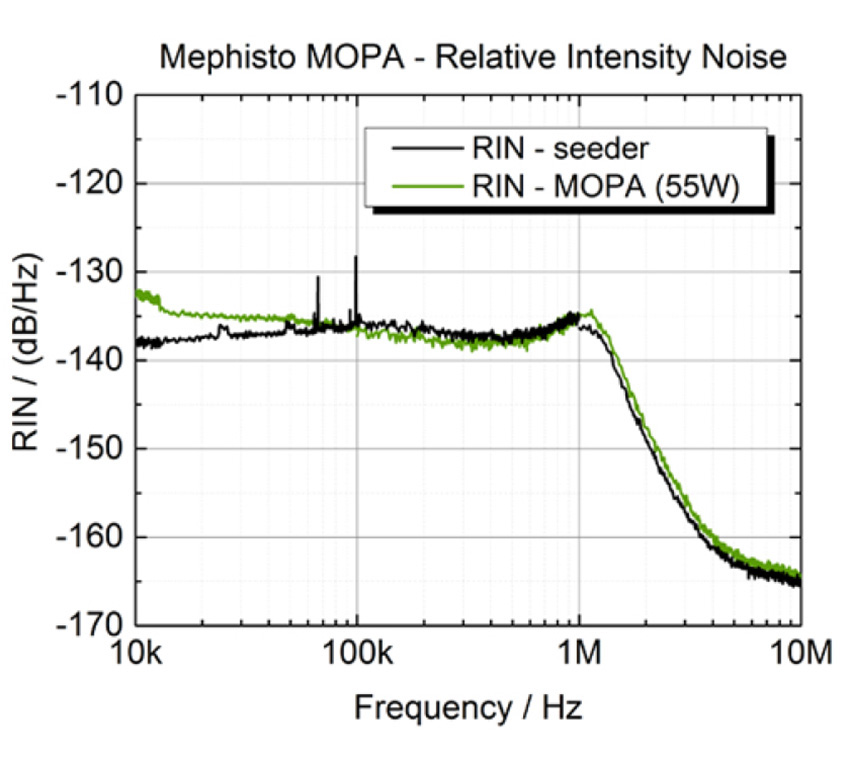
Fig. 7. Relative intensity noise (RIN) measured from the seed laser and the output of MOPA at a power of 55W
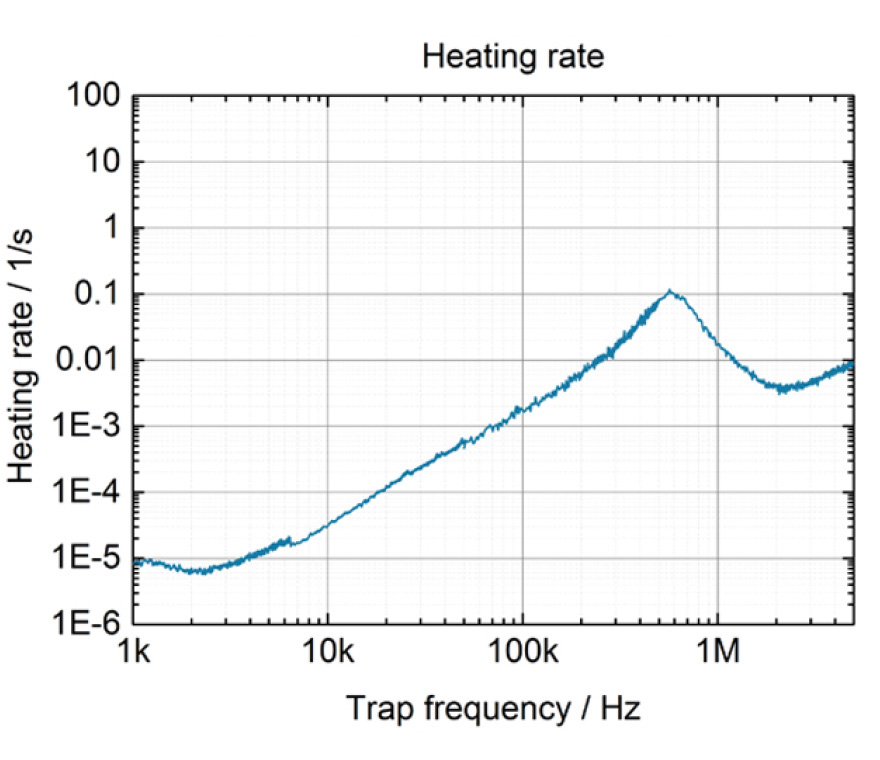
Fig. 8. Cold atoms heating rate due to laser intensity noise from the MOPA
Summary
Stable CW lasers are used in a number of different experimental methods for cold atom studies. Coherent Mephisto MOPA uses NPRO technology and well-established laser power amplification techniques to provide highly stable laser source which comes in a thoroughly field-tested, turnkey single-box solution. Ultra-narrow linewidth and market-leading phase and intensity stability parameters enable lowest noise and longest measurement times in experiments where optical dipole traps or optical lattices are used.