What are NonLinear Crystals?
Nonlinear crystals are specialized materials that interact with light in a way that changes its frequency (color), phase, polarization, and other properties. The magnitude of these effects is dependent upon the intensity of the input light. This is unlike traditional optics, where the interaction of light with the material doesn’t vary with the light’s intensity.
Nonlinear crystals are specialized materials that play a crucial role in the field of optics by enabling the manipulation of light in ways not possible with traditional optical materials. These crystals differ from their ‘linear’ counterparts by allowing the properties of light, such as its frequency, phase, and polarization, to be altered in response to the intensity of the light passing through them.
Nonlinear crystals are fundamental to a variety of advanced optical technologies and applications. These range from laser frequency conversion to optical telecommunication systems.
A detailed discussion of nonlinear crystals requires the use of advanced mathematics. Instead, this overview will provide a non-mathematical introduction to their basic principles and key applications, and highlight the most commonly used nonlinear crystal materials.
What are Nonlinear Optical Effects?
Most interactions between materials (whether solid, liquid, or gas) and light are linear. This means that the magnitude of the effect that the material has on light does not change with the light intensity. Thus, light/material interactions like refraction, reflection, transmission, absorption, and diffraction typically aren’t intensity dependent.
For example, the focal length of a lens, which is based on refraction, doesn’t change as the light gets brighter. The angle at which a mirror reflects light doesn’t vary with intensity.
The opposite is true of nonlinear optical effects. In this case, the intensity of the light influences how the material interacts with it. Sometimes this process is unwanted, but it can also be exploited to produce outcomes that cannot be achieved under linear conditions.
The most useful nonlinear effects are those which alter the frequency, amplify, or change the phase and/or polarization of light. The level of light intensity required to produce significant nonlinear effects is relatively high. Therefore, these effects are virtually negligible most of the time for most ordinary light sources. However, lasers can readily reach the required intensity levels, so the occurrence of nonlinear effects with them is relatively common. It’s worth looking at what each of these phenomena is individually.
Frequency Multiplication
Almost all high-power, industrial, solid-state lasers and fiber lasers emit near infrared light at around 1 µm in wavelength. However, it is advantageous in many materials processing applications to work at shorter wavelengths. This might be to better match the absorption of the material being processed – especially metals, which tend to be highly reflective in the infrared. Also, shorter wavelengths can be focused down into smaller spots. This enables the creation of smaller features.
Frequency conversion or multiplication is a widely used method for getting a shorter wavelength output from these various infrared lasers. For example, the 1064 nm output of a Nd:YVO₄ laser can be frequency doubled to 532 nm (green), or frequency tripled to 355 nm (ultraviolet). This is precisely how these output wavelengths are obtained in the Coherent AVIA LX, AVIA NX, and MATRIX 355 lasers. Frequency quadrupling of solid-state lasers to 266 nm (deeper into the ultraviolet) is possible, as well. Examples of this are the Coherent HyperRapid NXT and Azure NX.
How does this work? Frequency doubling, or second harmonic generation (SHG), happens when a nonlinear crystal converts a beam of light passing through it into exactly twice the original frequency (or half the wavelength). This occurs because the nonlinear nature of the crystal allows photons (light particles) in the beam to combine in pairs, transforming into single photons with double the energy. As a result, the color of the light changes to one that corresponds to the doubled frequency.
Frequency tripling takes the concept a step further by combining the effects of frequency doubling with an additional process to triple the light’s original frequency. This is typically achieved in two stages: first, the light is doubled in frequency, and then the doubled light is mixed with more of the original light within the same or a different nonlinear crystal. This interaction produces light with three times the energy (frequency) of the original light.
There are several conditions that must be met for this process to successfully occur. First, of course, the material itself must have the necessary ability to support nonlinear interactions with the incoming light. Second, the input laser light must have sufficient intensity; and the higher that intensity, the more efficient the operation of the nonlinear effect.
Another important condition for frequency multiplication is ‘phase matching.’ This need arises because dispersion within the nonlinear crystal causes the longer wavelength input light and the generated harmonic light to propagate at different velocities. This velocity mismatch can cause destructive interference between the two which reduces the efficiency of the harmonic generation.
Phase matching overcomes the challenge posed by dispersion. It aligns the phases of the fundamental and harmonic waves so that they propagate with the same effective velocity, maintaining constructive interference throughout the crystal. This alignment ensures that energy conversion from the fundamental wave to the harmonic wave is maximized.
Temperature control is also helpful for some nonlinear crystals. This is because temperature affects the crystal refractive index, which in turn influences phase matching conditions. This is why many manufacturers like Coherent offer their product integrated within a harmonic crystal oven.
Sum Frequency Generation and Difference Frequency Generation
Sum frequency generation (SFG) and difference frequency generation (DFG) are two other nonlinear processes that can change the wavelength of laser light. In these, two input light waves combine to produce a third light wave having a different frequency than the original beams. The key operating principle of SFG is that the frequency of the new light wave is the sum of the two input frequencies. Conversely, in DFG the new light wave frequency is the difference between the two input frequencies.
The optical parametric amplifier (OPA) is a particular embodiment of DFG used to amplify a laser beam without the need for the signal to be absorbed and then re-emitted by a medium. In an OPA, two light beams are introduced into the nonlinear crystal. One is a higher frequency, high-intensity ‘pump beam’ and the other is a lower frequency, low-power ‘signal beam’ (the one to be amplified). The properties of the nonlinear crystal allow energy from the pump beam to be transferred to the signal beam. This process called parametric down-conversion.
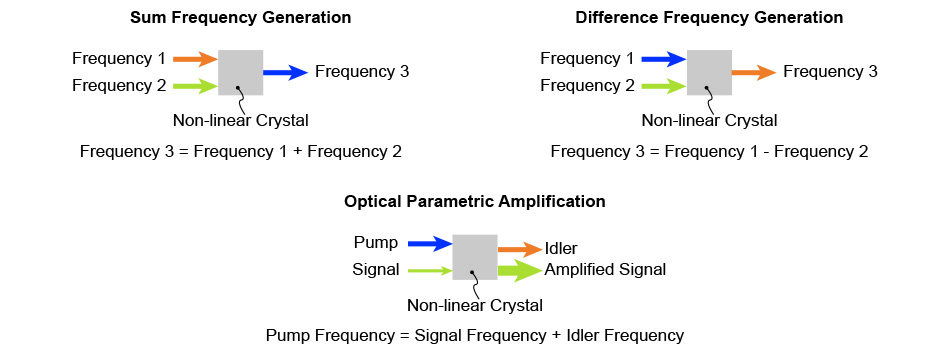
Schematic of SFG, DFG, and OPA, and the relationship between the input and output light frequencies in each.
The OPA delivers a number of benefits over traditional amplifiers. These includes low noise, typically better beam quality, pulse shaping capabilities, the ability to handle very high peak powers, and the ability to work with ultra-short pulses.
In addition to the amplified signal beam, the OPA also outputs an ‘idler beam.’ This is the DFG generated beam, so its frequency is the difference between the pump and signal beam frequencies.
This relationship also allows for wavelength tunability. In other words, it’s possible to both amplify the signal beam and select its frequency. The idler beam frequency must then change as well to keep the DFG conditions satisfied.
This can yield laser systems with extremely broad tunability, enabling them to serve a wide array of applications. For example, the Coherent OPerA Solo can tune of the enormous spectral range of 240 nm to 20 µm, depending upon exactly how it is configured.
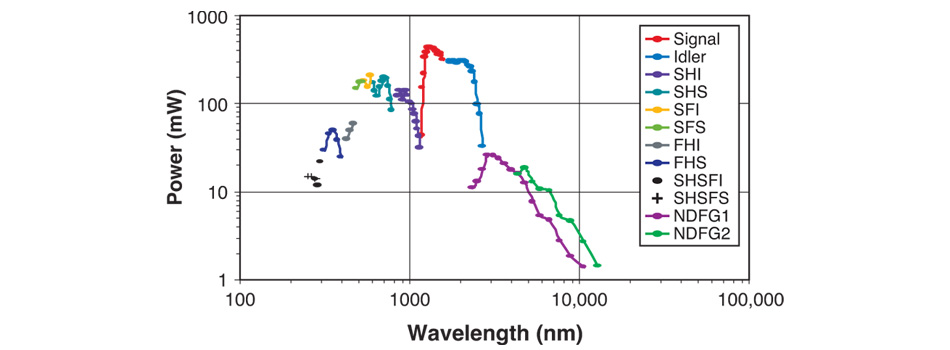
OPAs can such as the Coherent OPerA Solo provide a tremendous tuning range.
The Kerr Effect
The Kerr Effect is a nonlinear optical phenomenon in which the refractive index of a material changes in response to the intensity of the light passing through it. The more intense the light, the greater the change. The Kerr Effect enables modulation of light in real time, based on its intensity, and has numerous applications.
For example, the Kerr Effect is used in optical switches and modulators, which are crucial for telecommunications. By altering the intensity of light (and therefore the material refractive index), optical switches can control the direction of light in fiber-optic networks, enabling the routing of information at high speeds without converting to electrical signals.
Another use of the Kerr Effect is to shape laser pulses. Specifically, by using the Kerr Effect to produce a phase modulation, a pulse’s temporal and spectral characteristics can both be modified. This is vital for applications requiring precise control over laser pulse duration and frequency. Applications range from certain types of microscopy to materials processing.
The Kerr Effect also facilitates the formation of optical solitons. These are pulses of light that maintain their shape over long distances without dispersing. This property is particularly useful in long-distance fiberoptic communications, as solitons can carry information over vast distances with minimal loss or distortion.
Important Nonlinear Crystals
A variety of nonlinear crystals are currently in use. Each tends to be favored for a particular application (like SHG or OPA), or specific set of operating conditions. In general, however, the most popular materials are distinguished by their high nonlinear optical coefficients, wide transparency ranges, and good phase matching capabilities, along with various practical characteristics (available size, power handling capability, cost, etc.). Some of the most widely employed materials include the following:
Lithium Triborate (LBO): LBO is known for its high damage threshold and wide transparency range, making it suitable for high-power frequency doubling and OPO applications. It can be used for efficient SHG of both solid-state and other laser sources over a wide wavelength range.
Beta Barium Borate (BBO): BBO is favored for its wide transparency range (from the ultraviolet to the near infrared), high damage threshold, and high nonlinear optical coefficients. It is widely used for frequency doubling, tripling, and other nonlinear optical processes across a broad range of wavelengths, including in the ultraviolet region.
Potassium Titanyl Phosphate (KTP): KTP is often used for frequency doubling of solid-state lasers (at 1064 nm) to produce green light at 532 nm. It offers good nonlinear optical properties, a relatively high damage threshold, and is effective for OPO applications. KTP is also valued for its phase matching flexibility. In addition, KTP can periodically poled. This means creating periodic alternations in the orientation of its electric polarization. Periodic poling enables optical parametric modulation (OPM) and more efficient nonlinear interactions.
Potassium Dihydrogen Phosphate (KDP) and Potassium Dideuterium Phosphate (KD*P): These crystals are used for their high nonlinear optical coefficients and wide transparency range, particularly for frequency doubling and modulation of high-power lasers. They are also chosen for applications requiring large-aperture crystals due to their ease of growth in large sizes.
Lithium Niobate (LiNbO₃): Known for its strong electro-optic effect, lithium niobate is extensively used in modulators and for frequency doubling of near-infrared light. It has a wide transparency range and is capable of handling high power, but it requires high-intensity lasers for efficient SHG due to its relatively low nonlinear optical coefficient. LiNbO₃ can also be periodically poled.
Gallium Selenide (GaSe): GaSe is noted for its strong nonlinear optical responses in the mid-infrared to terahertz range, making it a preferred crystal for terahertz wave generation and mid-infrared applications.
AgGaS₂ and AgGaSe₂: These silver gallium sulfide and selenide crystals are important for mid-infrared applications, offering broad transparency ranges that extend into the mid-IR. They are particularly useful in parametric oscillators and for frequency mixing to generate mid-IR output.
Learn more about Coherent crystals.